October 22, 2012
by Artem Kaznatcheev
Last week, we saw how to deal with two-strategy games in finite inviscid populations. Unfortunately, two strategy games are not adequate to model many of the interactions we might be interested in. In particular, we cannot use Antal et al. (2009a) to look at bifurcations and finitary/stochastic effects in tag-based models of ethnocentrism, at least not without some subtle tricks. In this post we are going to look at a more general approach for any
-strategy game developed by Antal et al. (2009b). We will apply these tools to look at a new problem for the paper, but an old problem for the blog: ethnocentrism.
Antal et al. (2009b) consider a large but finite population of size
and a game
with
strategies. For update rule, they focus on the frequency dependent Moran process, although their results also hold for Wright-Fisher, and pairwise comparison (Fermi rule). Unlike the previous results for two-strategy games, the present work is applicable only in the limit of weak selection. Mutations are assumed to be uniform with probability
: a mutant is any one of the
strategies with equal probabilities. However, in section 4.2, the authors provide a cute argument for approximating non-uniform but parent-independent mutation rates by repeating strategies in proportion to the likelihood of mutation into them. Much like the two-strategy case, the authors derive separate equations for low and high mutation rates, and then provide a way to interpolate between the two extremes for intermediate mutation rates.
The mathematics proceeds through a perturbation analysis. The basic idea is to solve for the distribution of strategies in the drift (no selection) model, and then to gradually dial up the selection to perturb the distribution slightly into the weak selection regime. The authors use this to arrive at the following to conditions for low, and high mutation for strategy
to be favored:
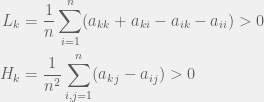
There is an intuitive story to help understand these two conditions. In the case of low mutation, the population is usually just two strategies until one of them fixes. So for
the terms that matter are the pairwise interactions between the strategies, and since the most decisive (and entropy rich) case is near the 50-50 split in the two strategies, self interactions (
) and other-strategy interactions (
) happen with the same frequency. I think this is where the discrepancy with Antal et. al (2009a) that we will see later sneaks in. A correction factor of
should be added in front of the the self-interaction terms, but I digress.
For the high mutation case, all the strategies are present in the population with about the same frequency at the same time. We need to look at the transitions from this population to get our first order terms. In that case, the focal individual’s fitness is
(since all opponents are equally likely; once again, I believe a correction term is in order), and the average fitness is
. The difference of these two terms produces
.
In order to interpolate, we have the following condition for strategy
to be more common than
for intermediate mutation rates
:

How does this disagree with the two-strategy results of Antal et al. (2009a)? The present paper reproduces the condition of risk-dominance, with C dominating D if
, but does not produce the small
correction of
. This would be mitigated with the observations I made earlier, but the approach of the perturbation analysis would have to be modified carefully.
The perturbation method can be extended to mixed strategies, as is done by Tarnita et al. (2009). In that case, we just replace the summation by integrals, to get:
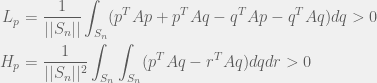
Where
is the n-vertex simplex with volume
. It is nice to know that the results generalize to mixed strategies, but not as important tool as the pure strategy variant. I will concentrate on pure strategies, although mixed might be good to revisit to study evolution of agency.
Antal et al. (2009b) showcase their method with three case studies: (i) cooperators, defectors, and loners, (ii) reversing the rank of strategies by mutation, and (iii) cooperators, defectors, and tit-for-tat. The first is the most interesting for me, since it shows how adding an irrelevant strategy, can reverse the dominance of the other two. I will present their example in a more general context for all cooperate-defect games. We will introduce an irrelevant strategy L, where irrelevance means that both C and D get the same payoff
from interacting with L and L gets
from them. The self interaction payoff for L can differ, and we can set it to
:

The authors consider the particular case of
and
, and
. We can apply the general results to get (for small mutations):
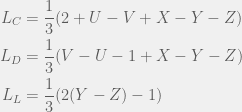
We can look at at the condition for C to dominate D for small mutations (
) to get
. If we had used
different irrelevant strategies (or just dialed up the proportion of mutations to an irrelevant strategy) then
would be replaced by
. This creates a new strip of cooperation which reaches into the Prisoner’s dilemma region (drawn in blue):

When we switch to large mutations, the region disappears and we recover the standard
rule. Note that this example means that, for this analysis, we cannot ignore competing strategies even if they are strictly dominated.
Ethnocentrism in tag-based models
We will consider a strategy space of
tags, with an agent of each tag being either a humanitarian (cooperate with all), ethnocentric (cooperate with same-tag), traitorous (cooperate with oot-tags), or selfish. For the game, we will look at the usual cost-benefit representation of Prisoner’s dilemma. Note that the
and
values will be the withing a single strategy of different tags, so we only need to compute four of each:
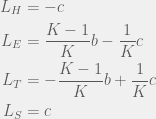
This divides the dynamics into two regions, when
then
, otherwise we have
. In other words, for large enough
or small enough
, ethnocentrism can be the dominant strategy in the population. This condition is in perfect agreement with the Traulsen & Nowak (2007) results we saw earlier. Although in that case, there were no H or T agents. If we remove H & T from the current analysis, we will still get the same condition for ethnocentric dominance even though we will calculate different
values.
For large mutations, the advantage of ethnocentrics disappears completely, and we get:
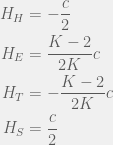
Which for
results in the ordering
. So if we have mutations that change tag and strategy together (as they do in this case) then higher mutation rates disadvantage the population, and if we let
be the expected number of mutants per generation, then we can see that ethnocentric cooperation is possible only if
or rewritten as
.
References
Antal, T., Nowak, M.A., & Traulsen, A. (2009a). Strategy abundance in games for arbitrary mutation rates Journal of Theoretical Biology, 257 (2), 340-344.
Antal T, Traulsen A, Ohtsuki H, Tarnita CE, & Nowak MA (2009b). Mutation-selection equilibrium in games with multiple strategies. Journal of Theoretical Biology, 258 (4), 614-22 PMID: 19248791
Tarnita, C.E., Antal, T., Nowak, M.A. (2009) Mutation-selection equilibrium in games with mixed strategies. Journal of Theoretical Biology 26(1): 50-57.
Traulsen A, & Nowak MA (2007). Chromodynamics of cooperation in finite populations. PLoS One, 2 (3).
Mutation-bias driving the evolution of mutation rates
March 31, 2016 by Julian Xue 4 Comments
In classic game theory, we are often faced with multiple potential equilibria between which to select with no unequivocal way to choose between these alternatives. If you’ve ever heard Artem justify dynamic approaches, such as evolutionary game theory, then you’ve seen this equilibrium selection problem take center stage. Natural selection has an analogous ‘problem’ of many local fitness peaks. Is the selection between them simply an accidental historical process? Or is there a method to the madness that is independent of the the environment that defines the fitness landscape and that can produce long term evolutionary trends?
Two weeks ago, in my first post of this series, I talked about an idea Wallace Arthur (2004) calls “developmental bias”, where the variation of traits in a population can determine which fitness peak the population evolves to. The idea is that if variation is generated more frequently in a particular direction, then fitness peaks in that direction are more easily discovered. Arthur hypothesized that this mechanism can be responsible for long-term evolutionary trends.
A very similar idea was discovered and called “mutation bias” by Yampolsky & Stoltzfus (2001). The difference between mutation bias and developmental bias is that Yampolsky & Stoltzfus (2001) described the idea in the language of discrete genetics rather than trait-based phenotypic evolution. They also did not invoke developmental biology. The basic mechanism, however, was the same: if a population is confronted with multiple fitness peaks nearby, mutation bias will make particular peaks much more likely.
In this post, I will discuss the Yampolsky & Stoltzfus (2001) “mutation bias”, consider applications of it to the evolution of mutation rates by Gerrish et al. (2007), and discuss how mutation is like and unlike other biological traits.
Read more of this post
Filed under Commentary, Models, Reviews Tagged with evolution, mutation, supply driven evolution